CERN is home to a unique centre-of-excellence in vacuum science, technology and engineering. Paolo Chiggiato and Luigi Scibile explain how that collective expertise is being put to work as part of the international effort to develop the next generation of gravitational-wave telescopes.
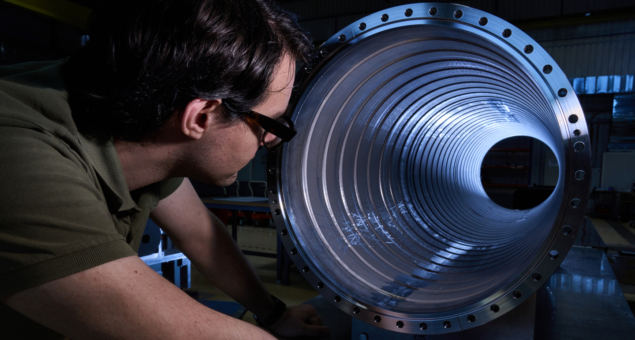
The first detection of gravitational waves in 2015 stands as a confirmation of Einstein’s prediction in his general theory of relativity and represents one of the most significant milestones in contemporary physics. Not only that, direct observation of gravitational ripples in the fabric of space-time opened up a new window on the universe that enables astronomers to study cataclysmic events such as black-hole collisions, supernovae and the merging of neutron stars. The hope is that the emerging cosmological data sets will, over time, yield unique insights to address fundamental problems in physics and astrophysics – the distribution of matter in the early universe, for example, and the search for dark matter and dark energy.
By contrast, an altogether more down-to-earth agenda – Beampipes for Gravitational Wave Telescopes 2023 – provided the backdrop for a three-day workshop held at CERN at the end of March. Focused on enabling technologies for current and future gravitational-wave observatories – specifically, their ultrahigh-vacuum (UHV) beampipe requirements – the workshop attracted a cross-disciplinary audience of 85 specialists drawn from the particle-accelerator and gravitational-wave communities alongside industry experts spanning steel production, pipe manufacturing and vacuum technologies (CERN Courier July/August 2023 p18).
If location is everything, Geneva ticks all the boxes in this regard. With more than 125 km of beampipes and liquid-helium transfer lines, CERN is home to one of the world’s largest vacuum systems – and certainly the longest and most sophisticated in terms of particle accelerators. All of which ensured a series of workshop outcomes shaped by openness, encouragement and collaboration, with CERN’s technology and engineering departments proactively sharing their expertise in vacuum science, materials processing, advanced manufacturing and surface treatment with counterparts in the gravitational-wave community.
Measurement science
To put all that knowledge-share into context, however, it’s necessary to revisit the basics of gravitational-wave metrology. The principal way to detect gravitational waves is to use a laser interferometer comprising two perpendicular arms, each several kilometres long and arranged in an L shape. At the intersection of the L, the laser beams in the two branches interact, whereupon the resulting interference signal is captured by photodetectors. When a gravitational wave passes through Earth, it induces differential length changes in the interferometer arms – such that the laser beams traversing the two arms experience dissimilar path lengths, resulting in a phase shift and corresponding alterations in their interference pattern.
Better by design: the Einstein Telescope beampipes
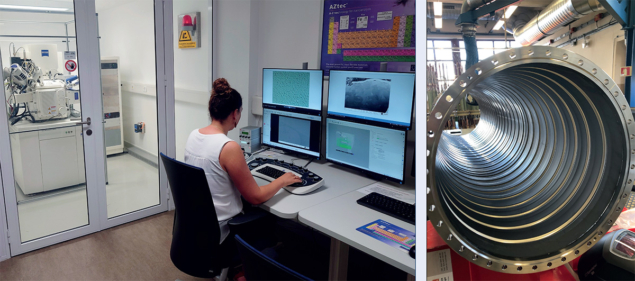
The baseline for the Einstein Telescope’s beampipe design studies is the Virgo gravitational-wave experiment. The latter’s beampipe – which is made of austenitic stainless steel (AISI 304L) – consists of a 4 mm thick wall reinforced with stiffener rings and equipped with an expansion bellows (to absorb shock and vibration).
While steel remains the material of choice for the Einstein Telescope beampipe, other grades beyond AISI 304L are under consideration. Ferritic steels, for example, can contribute to a significant cost reduction per unit mass compared to austenitic stainless steel, which contains nickel. Ferrite also has a body-centred-cubic crystallographic structure that results in lower residual hydrogen levels versus face-centred-cubic austenite – a feature that eliminates the need for expensive solid-state degassing treatments when pumping down to UHV.
Options currently on the table include the cheapest ferritic steels, known as “mild steels”, which are used in gas pipelines after undergoing corrosion treatment, as well as ferritic stainless steels containing more than 12% chromium by weight. While initial results with the latter show real promise, plastic deformation of welded joints remains an open topic, while the magnetic properties of these materials must also be considered to prevent anomalous transmission of electromagnetic signals and induced mechanical vibrations.
Along a related coordinate, CERN is developing an alternative solution with respect to the “baseline design” that involves corrugated walls with a thickness of 1.3 mm, eliminating the need for bellows and reinforcements. Double-wall pipe designs are also in the mix – either with an insulation vacuum or thermal insulators between the two walls.
Beyond the beampipe material, studies are exploring the integration of optical baffles, which intermittently reduce the pipe aperture to block scattered photons. Various aspects such as positioning, material, surface treatment and installation are under review, while the transfer of vibrations from the tunnel structure to the baffle represents another line of enquiry.
With this in mind, the design of the beampipe support system aims to minimise the transmission of vibrations to the baffles and reduce the frequency of the first vibration eigen mode within a range where the Einstein Telescope is expected to be less sensitive. Defining the vibration transfer function from the tunnel’s near-environment to the beampipe is another key objective, as are the vibration levels induced by airflow in the tunnel (around the beampipe) and stray electromagnetic fields from beampipe instrumentation.
Another thorny challenge is integration of the beampipes into the Einstein Telescope tunnel. Since the beampipes will be made up of approximately 15 m-long units, welding in the tunnel will be mandatory. CERN’s experience in welding cryogenic transfer lines and magnet junctions in the LHC tunnel will be useful in this regard, with automatic welding and cutting machines being one possible option to streamline deployment.
Also under scrutiny is the logistics chain from raw material to final installation. Several options are being evaluated, including manufacturing and treating the beampipes on-site to reduce storage needs and align production with the pace of installation. While this solution would reduce the shipping costs of road and maritime transport, it would require specialised production personnel and dedicated infrastructure at the Einstein Telescope site.
Finally, the manufacturing and treatment processes of the beampipes will have a significant impact on cost and vacuum performance – most notably with respect to dust control, an essential consideration to prevent excessive light scattering due to falling particles and changes in baffle reflectivity. Dust issues are common in particle accelerators and the lessons learned at CERN and other facilities may well be transferable to the Einstein Telescope initiative.
These are no ordinary interferometers, though. The instruments operate at the outer limits of measurement science and are capable of tracking changes in length down to a few tens of zeptometres (10–21 m), a length scale roughly 10,000 times smaller than the diameter of a proton. This achievement is the result of extraordinary progress in optical technologies over recent decades – advances in laser stability and mirror design, for example – as well as the ongoing quest to minimise sources of noise arising from seismic vibrations and quantum effects.
With the latter in mind, the interferometer laser beams must also propagate through vacuum chambers to avoid potential scattering of the light by gas molecules. The residual gas present within these chambers introduces spatial and temporal fluctuations in the refractive index of the medium through which the laser beam propagates – primarily caused by statistical variations in gas density.
As such, the coherence of the laser beam can be compromised as it traverses regions characterised by a non-uniform refractive index, resulting in phase distortions. To mitigate the detrimental effects of coherence degradation, it is therefore essential to maintain hydrogen levels at pressures lower than 10–9 mbar, while even stricter UHV requirements are in place for heavier molecules (depending on their polarisability and thermal speed).
Now and next
Right now, there are four gravitational-wave telescopes in operation: LIGO (across two sites in the US), Virgo in Italy, KAGRA in Japan, and GEO600 in Germany (while India has recently approved the construction of a new gravitational-wave observatory in the western state of Maharashtra). Coordination is a defining feature of this collective endeavour, with the exchange of data among the respective experiments crucial for eliminating local interference and accurately pinpointing the detection of cosmic events.
Meanwhile, the research community is already planning for the next generation of gravitational-wave telescopes. The primary objective: to expand the portion of the universe that can be comprehensively mapped and, ultimately, to detect the primordial gravitational waves generated by the Big Bang. In terms of implementation, this will demand experiments with longer interferometer arms accompanied by significant reductions in noise levels (necessitating, for example, the implementation of cryogenic cooling techniques for the mirrors).
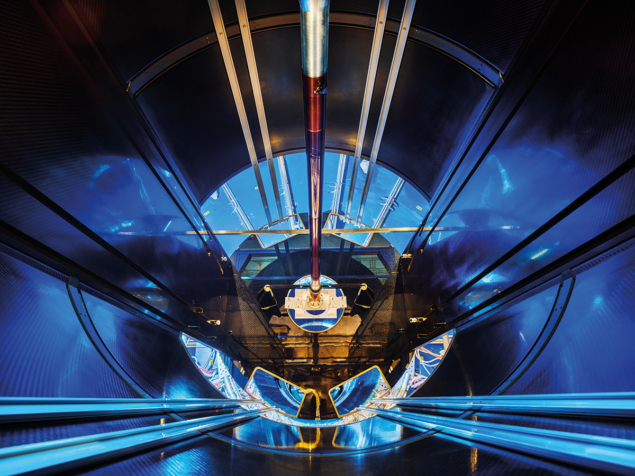
Two leading proposals are on the table: the Einstein Telescope in Europe and the Cosmic Explorer in the US. The latter proposes a 40 km long interferometer arm with a 1.2 m diameter beampipe, configured in the traditional L shape and across two different sites (as per LIGO). Conversely, the former proposes six 60° Ls in an underground tunnel laid out in an equilateral triangle configuration (10 km long sides, 1 m beampipe diameter and with a high- and low-frequency detector at each vertex).
For comparison, the current LIGO and Virgo installations feature arm lengths of 4 km and 3 km, respectively. As a result, the anticipated length of the vacuum vessel for the Einstein Telescope is projected to be 120 km, while for the Cosmic Explorer it is expected to be 160 km. In short: both programmes will require the most extensive and ambitious UHV systems ever constructed.
Extreme vacuum
At a granular level, the vacuum requirements for the Einstein Telescope and Cosmic Explorer assume that the noise induced by residual gas is significantly lower than the allowable noise budget of the gravitational interferometers themselves. This comparison is typically made in terms of amplitude spectral density. A similar approach is employed in particle accelerators, where an adequately low residual gas density is imperative to minimise any impacts on beam lifetimes (which are predominantly constrained by other unavoidable factors such as beam-beam interactions and collimation).
The specification for the Einstein Telescope states that the contribution of residual gas density to the overall noise budget must not exceed 10%, which necessitates that hydrogen partial pressure be maintained in the low 10–10 mbar range. Achieving such pressures is commonplace in leading-edge particle accelerator facilities and, as it turns out, not far beyond the limits of current gravitational-wave experiments. The problem, though, comes when mapping current vacuum technologies to next-generation experiments like the Einstein Telescope.
In such a scenario, the vacuum system would represent one of the biggest capital equipment costs – on a par, in fact, with the civil engineering works (the main cost-sink). As a result, one of the principal tasks facing the project teams is the co-development – in collaboration with industry – of scalable vacuum solutions that will enable the cost-effective construction of these advanced experiments without compromising on UHV performance and reliability.
Follow the money
It’s worth noting that the upward trajectory of capital/operational costs versus length of the experimental beampipe is a challenge that’s common to both next-generation particle accelerators and gravitational-wave telescopes – and one that makes cost reduction mandatory when it comes to the core vacuum technologies that underpin these large-scale facilities. In the case of the proposed Future Circular Collider at CERN, for instance, a vacuum vessel exceeding 90 km in length would be necessary.
Of course, while operational and maintenance costs must be prioritised in the initial design phase, the emphasis on cost reduction touches all aspects of project planning and, thereafter, requires meticulous optimisation across all stages of production – encompassing materials selection, manufacturing processes, material treatments, transport, logistics, equipment installation and commissioning. Systems integration is also paramount, especially at the interfaces between the vacuum vessel’s technical systems and adjacent infrastructure (for example, surface buildings, underground tunnels and caverns). Key to success in every case is a well-structured project that brings together experts with diverse competencies as part of an ongoing “collective conversation” with their counterparts in the physics community and industrial supply chain.
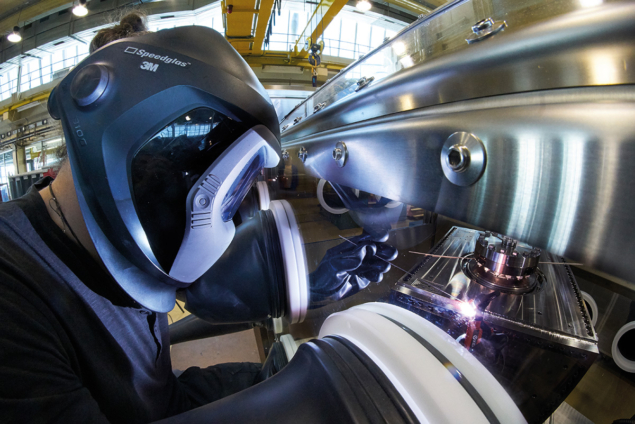
Within this framework, CERN’s specialist expertise in managing large-scale infrastructure projects such as the HL-LHC can help to secure the success of future gravitational-wave initiatives. Notwithstanding CERN’s capabilities in vacuum system design and optimisation, other areas of shared interest between the respective communities include civil engineering, underground safety and data management, to name a few.
Furthermore, such considerations align well with the latest update of the European strategy for particle physics – which explicitly prioritises the synergies between particle and astroparticle physics – and are reflected operationally through a collaboration agreement (signed in 2020) between CERN and the lead partners on the Einstein Telescope feasibility study – Nikhef in the Netherlands and INFN in Italy.
In this way, CERN is engaged directly as a contributing partner on the beampipe studies for the Einstein Telescope (see “Better by design: the Einstein Telescope beampipes”). The three-year project, which kicked off in September 2022, will deliver the main technical design report for the telescope’s beampipes. CERN’s contribution is structured in eight work packages, from design and materials choice to logistics and installation, including surface treatments and vacuum systems.
CERN teams are engaged directly on the beampipe studies for the Einstein Telescope
The beampipe pilot sector will also be installed at CERN, in a building previously used for testing cryogenic helium transfer lines for the LHC. Several measurements are planned for 2025, including tests relating to installation, alignment, in-situ welding, leak detection and achievable vacuum levels. Other lines of enquiry will assess the efficiency of the bakeout process, which involves the injection of electrical current directly into the beampipe walls (heating them in the 100–150 °C range) to minimise subsequent outgassing levels under vacuum.
Given that installation of the beampipe pilot sector is time-limited, while details around the manufacturing and treatment of the vacuum chambers are still to be clarified, the engagement of industry partners in this early design stage is a given – an approach, moreover, that seeks to replicate the collaborative working models pursued as standard within the particle-accelerator community. While there’s a lot of ground to cover in the next two years, the optimism and can-do mindset of all participants at Beampipes for Gravitational Wave Telescopes 2023 bodes well.